While manipulating the internal states of atoms with electromagnetic fields is enormously powerful, these fields can also carry momentum, which, because of matter-wave duality, can be transferred to the atom. The complementarity between the discoveries of de Broglie and Compton mean that when atoms absorb a photon, conservation of momentum results in a change in the atom’s motion.
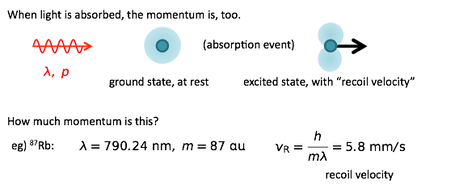
The magnitude of this velocity, ~ 6 mm/s in the case of rubidium, is on the scale of the speeds associated with the low temperatures needed to reach the quantum mechanical regime discussed earlier, and so, researchers have found ways to manipulate the motion of atoms using light, the first kind of transition discussed previously.
One of the important features of these atoms is the specificity of the laser transitions -- they are very precise. One must use lasers whose wavelengths are very well controlled to match the energy level differences between the atomic states. These energies and wavelengths are so specific that the Doppler effect is important -- atoms that are moving see slightly different wavelengths than those at rest.
Absorption and spontaneous emission
Two processes are important to consider for laser cooling. The first, absorption, excites the atoms from its ground to an excited state. The resulting motion of the atom is in the direction of the photon’s original momentum. The second process is spontaneous emission -- because of interactions with the vacuum, the atom relaxes to its ground state in about 30 ns. The photon that is expelled will do so in a random direction, giving the atom a momentum kick in the opposite direction. Because of the randomness of this process, on average, the net momentum of both these processes is half of a recoil of momentum in the direction of the original photons. When many of these absorption-emission events happen, the atom’s velocity will be tend towards the direction of the absorbing photons’.
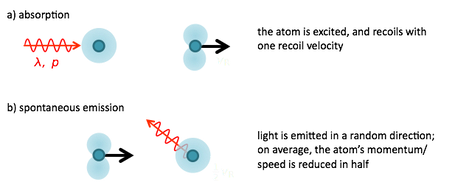
The Doppler effect
One of the important features of these atoms is the specificity of the laser transitions -- they are very precise. One must use lasers whose wavelengths are very well controlled to match the energy level differences between the atomic states. These energies and wavelengths are so specific that the Doppler effect is important -- atoms that are moving see slightly different wavelengths than those at rest.
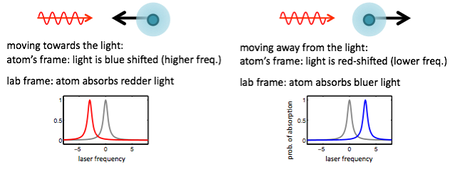
The Zeeman effect
Another way we can control the energy of transitions between different internal levels of the atoms is with magnetic fields — using the “Zeeman effect.” If we set up a magnetic field that has gradients — different values at different points in space — the atom’s energy will depend upon its spatial location.
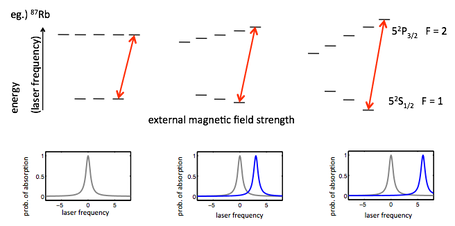
The Zeeman slower
To best manipulate the motion of the atoms, we use both the Doppler and Zeeman effects to our advantage. The Zeeman slower is a device used in many experiments to implement pre-cooling of an atomic beam along one dimension.
The basic principles are this: a tapered solenoid is constructed so that the magnetic field, pointing along the direction of the solenoid, is large at one end (in the diagram below, on the right side) and small at the other (left). Hot, fast moving atoms enter the large-field region, and a laser beam with momentum opposing the motion of the atoms is sent in from the opposite direction. In the high field region, the energy levels are pushed apart, away from resonance with the light. However, the atoms are moving fast, and the light they see is Doppler shifted to higher frequencies, such that it is on resonance in the high field region. The atoms thus absorb photons from the beam and slow down. As they slow down and continue to move into a lower-field region, the Zeeman shift is reduced, but so is the Doppler shift. With proper design of the magnetic fields, the atoms will stay on resonance as they move down the solenoid with the chance to absorb many photons along the way and have their momentum greatly reduced. This is the principle underlying laser cooling.
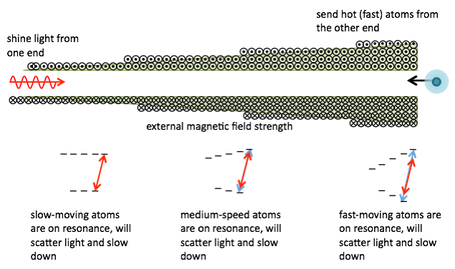
In experiments, all three dimensions must be cooled. This is accomplished via a “magneto-optical trap,” which has a magnetic field designed to vary in all three dimensions, and counter-propagating laser beams impinging along all three axes. The magnetic field (magnitude in orange below) is usually generate by a pair of anti-Helmholtz coils and has a local minimum and increases away from the centre of the system. Red-detuned laser beams shine from all three directions in such a way as to be preferrentially absorbed by any atoms moving towards them, impelling atoms to return to the centre of the system, with smaller velocities. This powerful cooling technique allows atoms to reach (in the case of rubidium) tens of microKelvin.
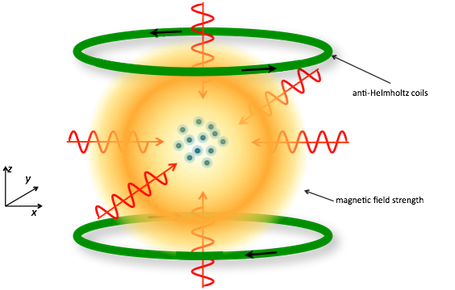
In addition, more complicated mechanisms that exploit the details of the atoms’ internal states and the polarization of the light allow researchers to reach even colder temperatures than are allowed by the Doppler cooling mechanism explained above.
Related to all this, I made a short movie talking about the misconception that lasers always make things hot....
This browser cannot play the embedded video file.